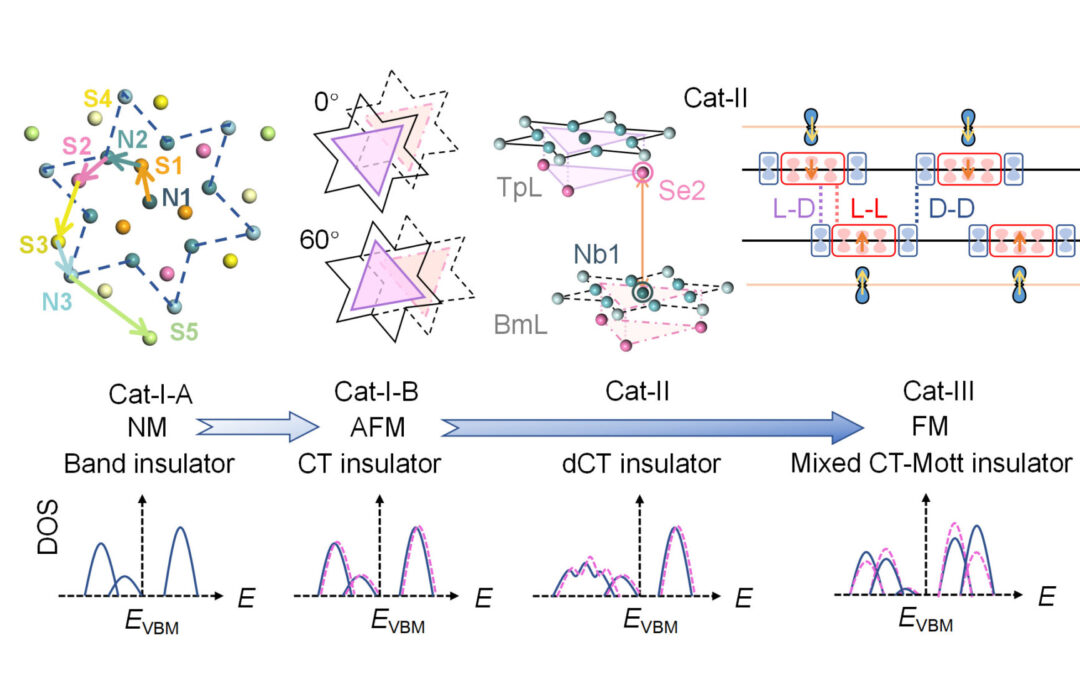
NbSe2

Arxiv
Layer sliding and twisting induced electronic transitions in correlated magnetic 1T-NbSe2 bilayers
Mott and CT insulators are representative materials, like 1T-phases of TaS2, TaSe2 and NbSe2. In the strong-correlation limit of electron correlated systems, on-site Coulomb interactions split a half-filled band into two sub-bands, namely the lower (LHB) and upper Hubbard bands (UHB), forming a Mott or a CT insulator[13]. The governing coupling mechanism lies in the interlayer electronic hybridization of interfacial Se pz orbitals within a localized region of the David star, rather than previously supposed metal atoms dz2 orbitals. Subtle differences in interlayer hybridization vary the energy levels of the four Hubbard bands in the 1T-NbSe2 bilayer. Three electronic and two magnetic transitions among four insulating states were observed upon interlayer sliding or twisting, while three of the four insulating states are correlated ones. All these striking results highlight the importance of interlayer coupling in tunning correlated electronic states in NbSe2 bilayers.

Mirror twin boundaries (MTBs)[14,15] was demonstrate to be another strategy to introduce additional exotic electronic states in chalcogen-deficient 1H-MoS2[16], -MoSe2[14], and – MoTe2[17]monolayers. In some lattices with specific symmetries, such as kagome lattice, the intrinsic flat band leads to a high density of electron states. A TMD layer consisting of ordered and uniformly sized MTB triangles, namely an MTB-triangle lattice, could be a TMD phase exhibiting a well-defined lattice symmetry. Coloring-triangular (CT) lattice[18] in a MoTe2 (CT-MoTe2) monolayer comprise of uniform-sized and orderly arranged MTB triangles and normal MoTe2 domains embedded among MTBs. Dirac-like and flat electronic bands inherently existing in the CT lattice are identified by two broad and two prominent peaks. Further more, the CT-MoTe2 monolayer shows energy-dependent electronic Janus lattices, including the original atomic-lattice and an electronic Te pseudo-sublattice.
1. Zhang H, et al. Tailored Ising superconductivity in intercalated bulk NbSe2. Nature Physics 18, 1425-1430 (2022).
2. Cao Y, et al. Unconventional superconductivity in magic-angle graphene superlattices. Nature 556, 43-50 (2018)
3. Klanjšek M, et al. A high-temperature quantum spin liquid with polaron spins. Nature Physics 13, 1130-1134 (2017)
4. Law KT, Lee PA. 1T-TaS2 as a quantum spin liquid. Proceedings of the National Academy of Sciences 114, 6996-7000 (2017)
5. Chen Y, et al. Strong correlations and orbital texture in single-layer 1T-TaSe2. Nature Physics 16, 218-224 (2020)
6. Liu M, et al. Monolayer 1T-NbSe2 as a 2D-correlated magnetic insulator. Science Advances 7, eabi6339 (2021)
7. Wang YD, et al. Band insulator to Mott insulator transition in 1T-TaS2. Nature Communications 11, 4215 (2020)
8. Grasset R, et al. Pressure-induced collapse of the charge density wave and Higgs mode visibility in 2H− TaS2. Physical Review Letters 122, 127001 (2019)
9. Lian C-S, Si C, Duan W. Unveiling Charge-Density Wave, Superconductivity, and Their Competitive Nature in Two-Dimensional NbSe2. Nano Letters 18, 2924-2929 (2018)
10. Li H, et al. Imaging two-dimensional generalized Wigner crystals. Nature 597, 650-654 (2021)
11. Regan EC, et al. Mott and generalized Wigner crystal states in WSe2/WS2 moiré superlattices. Nature 579, 359-363 (2020)
12. Zhou Y, et al. Bilayer Wigner crystals in a transition metal dichalcogenide heterostructure. Nature 595, 48-52 (2021)
13. Zaanen J, Sawatzky GA, Allen JW. Band gaps and electronic structure of transition-metal compounds. Physical Review Letters 55, 418-421 (1985)
14. Liu H, et al. Dense Network of One-Dimensional Midgap Metallic Modes in Monolayer MoSe2 and Their Spatial Undulations. Physical Review Letters 113, 066105 (2014)
15. Hong J, et al. Inversion Domain Boundary Induced Stacking and Bandstructure Diversity in Bilayer MoSe2. Nano Letters 17, 6653-6660 (2017)
16. Zhou W, et al. Intrinsic Structural Defects in Monolayer Molybdenum Disulfide. Nano Letters 13, 2615-2622 (2013)
17. Diaz HC, Ma Y, Chaghi R, Batzill M. High density of (pseudo) periodic twin-grain boundaries in molecular beam epitaxy-grown van der Waals heterostructure: MoTe2/MoS2. Applied Physics Letters 108, 191606 (2016)
18. Zhang S, et al. Kagome bands disguised in a coloring-triangle lattice. Physical Review B 99, 100404 (2019)